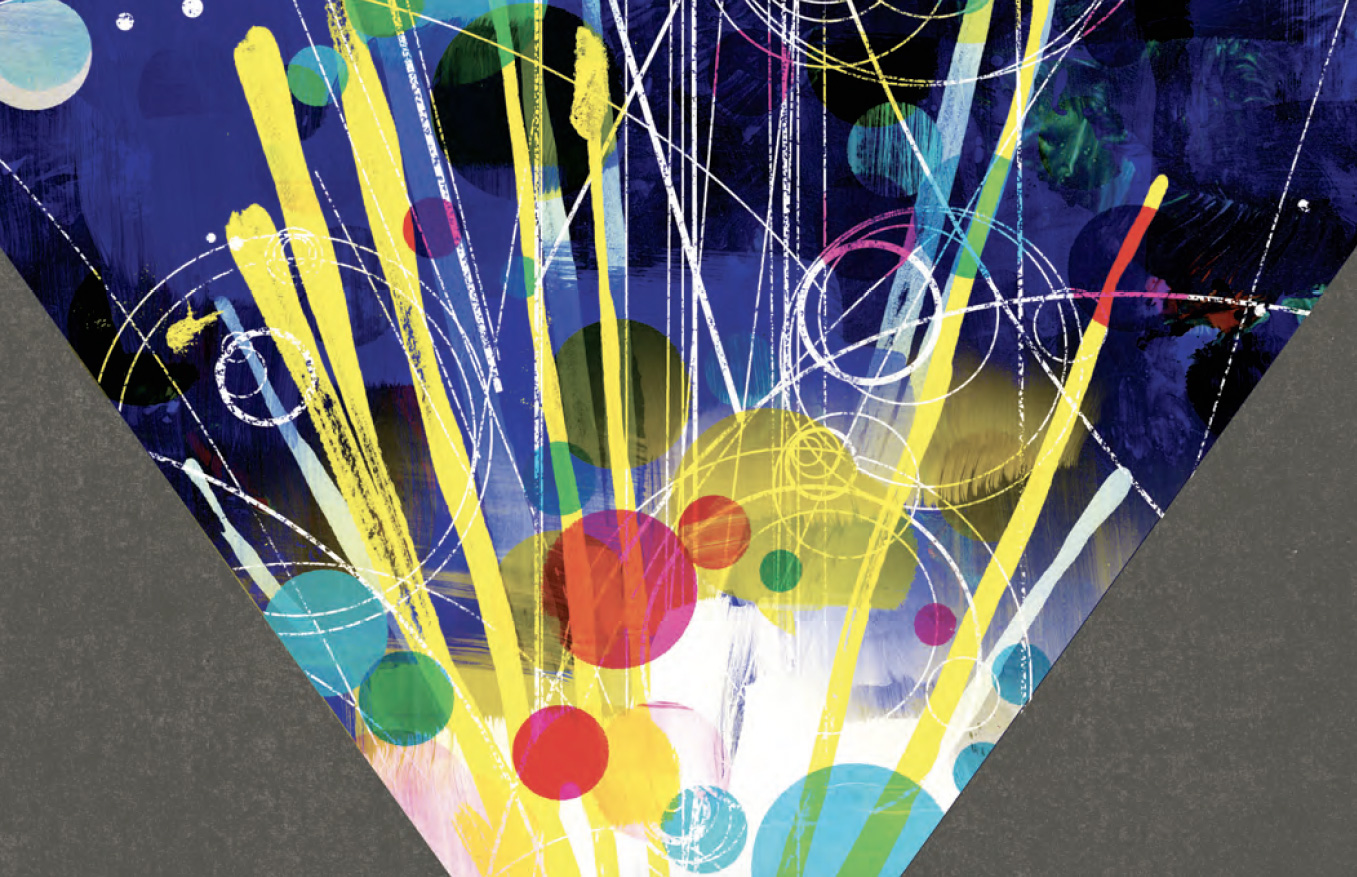
John Parsons is poised to enter a new dimension.
It is a cloudy Monday morning in April, and the fifty-year-old physics professor is sitting in a swivel chair in his Pupin Hall office with his face inches away from his computer. On the screen is a colorful chart showing what happened when two protons zipped around the seventeen-mile-long circular tunnel of the world’s most powerful particle accelerator, the Large Hadron Collider (LHC), outside Geneva, and smashed together at nearly the speed of light. When the protons met, the immense energy that had been stored up in their motion was suddenly released in the form of quarks, photons, electrons, gluons, muons, pions, kaons, and other particles that materialized like tornadoes churned out by a furious weather system. The new particles spun outward in all directions, each one moving in a manner that revealed its personality: some made a beeline for the collider’s outer wall, others floated gently like streamers, and still others spiraled, as if trying to return from where they came. Their journeys, which took less than a billionth of a second and were tracked by computerized sensors, now made for a staggeringly complex and beautiful-looking puzzle.
Within the tangle, Parsons zeroes in on a single particle. He can tell it is a photon, or a light particle, by the clean angle with which it hit the detector. Photons are the fastballs of the particle world, always firing straight and hard. Parsons hopes that this one is pointing him to one of the most extraordinary scientific discoveries in a century.
“Look at where it came from,” Parsons says, zooming in on the image. “Not from the point of the original collision, but a few centimeters off.” The photon seems to have come from nowhere. This is a sign that it’s a byproduct of another particle that was produced by the collision and then quickly decayed and disappeared. What could that be? “One possibility is a neutralino,” Parsons says. “And where there’s a neutralino, there may also be a gravitino.”
The gravitino is precious quarry for experimental physicists: a new particle whose discovery would radically alter our understanding of nature. Since the 1970s, the so-called Standard Model of particle physics has been the law of the cosmos; its seventeenth and final cog, the Higgs boson, was found last year. A gravitino would be a different sort of beast entirely. This little ball of thunder would provide the first evidence for a controversial idea called supersymmetry, which holds that each of the seventeen particles already identified has a nearly identical cousin still hiding in the shadows: the photon gets a photino, the electron a selectron, each of six types of quark a squark, each of eight types of gluon a gluino, and so on. The theory goes that these supersymmetrical particles, or sparticles, are the missing puzzle pieces needed to solve mysteries that the Standard Model doesn’t even try to address, mysteries such as: What came before the Big Bang? How does gravity work? Why is our universe expanding?
“If supersymmetry is right, we’re basically talking about the key to a unified theory of everything,” Parsons says. “The thought of it is thrilling.”
If he is to find a gravitino, Parsons will need to be a creative sleuth. This is because a gravitino is thought to be impervious to electromagnetism and other forces. It is, in other words, invisible. Whereas most particles that pass through the LHC’s silicon, liquid-argon, and iron sensors will knock electrons loose from their atoms and leave an irradiated trail of ions in their stead, a gravitino will slip through these heavy materials as if they were cheesecloth.
How do you discover something you can’t see?
“You look for curious absences,” says Parsons, who has been conducting the analysis with Columbia graduate student Nikiforos Nikiforou and physicists at the University of Liverpool. “First, you’ll see less energy coming out of a collision than went into it, which suggests that something is sneaking past your sensors on the way out. And perhaps you’ll notice these little oddities in the direction of your photons.”
Parsons, a rosy-skinned Canadian with a calm demeanor, believes he has as good a chance as anybody of finding a sparticle. After all, he has spent the past twenty years designing, testing, and calibrating the circuit boards that act as the electronic brains in the LHC’s largest detector, known as ATLAS. He did this work with Bill Willis, a Columbia professor who died this past fall and who was among the first prominent American physicists to get involved in the European-led LHC project, in the early 1990s.
“If you spend two decades developing a piece of machinery, you really get to know it,” Parsons says. “You know its strengths, its limitations, and its quirks. And this gives you an intuitive feel for working with the data.”
Over the past few years, Parsons and Willis used their intimate knowledge of the ATLAS detector to develop new analytic techniques that enabled scientists for the first time to identify photons that are second-generation byproducts of collisions. The method involves not only tracing the photons’ paths but also recording how much time they take to reach the detector’s sensors: even the slightest delay will signal that they sprouted from other, slower particles that traveled the first few centimeters from the collision.
Parsons and Willis’s techniques were instrumental in finding the Higgs boson, which had been a Holy Grail for physicists since being theorized in the 1960s. The Higgs is not invisible, although it might as well be: it appears only fleetingly in high-energy circumstances before transmuting into more ordinary particles like photons, the way a hurricane downgrades into a tropical storm.
“One of the Higgs’s most distinctive decay patterns is turning into two photons,” Parsons says. “So analyzing the journeys of these light particles was helpful in pulling off its mask.”
The importance of the Higgs boson’s discovery, which was announced last summer, is difficult to overstate. The Higgs is a physical manifestation of an energy field that permeates space and acts like cosmic molasses, slowing down particles as they move. It thus explains how particles acquire mass, which was the last remaining gap in the Standard Model.
To Parsons and to many other scientists working at the Large Hadron Collider, however, the real drama is just beginning. As important as the discovery of the Higgs boson was, it hardly came as a surprise. The existence of the Higgs had been theorized for so long, and physicists had accumulated so much indirect evidence for it, that the work had come to feel like a long, slow march toward the inevitable.
“There is an entire generation of physicists, of which I’m a part, who have spent their careers with the Higgs looming over the horizon,” says Parsons. “It is satisfying to have finished the job. But frankly, we’re also happy to move on to other things. The search for supersymmetry is exciting because nobody knows if it’s real or not. Finding it would be a lightning bolt.”
Into the Weird
The concept of supersymmetry grew out of physicists’ attempts in the 1970s and ’80s to resolve a paradox they had observed in nature: that tiny objects and larger ones are controlled by different forces. In the quantum world, activity between particles is governed by electromagnetism and the so-called weak and strong forces that bind atoms together. Gravity is imperceptibly weak in this realm — so weak, in fact, that particle physicists don’t even figure it into their equations. Yet the interactions of more massive bodies — from dust particles, say, all the way up to planets, stars, and galaxies — are dominated by gravity.
“It turns out that our best descriptions of the quantum world and the macroscale world just don’t mix,” says Brian Greene, a Columbia theoretical physicist and mathematician. “And if you’re in the business of trying to identify truly universal laws to describe nature, that’s a problem.”
Supersymmetry’s solution is to propose that the differences between the forces are not fundamental or irreconcilable at all but merely seem this way because of the limitations of our perspective — namely, that we are stuck observing a universe that has been cooling and expanding for fourteen billion years. If only we could glimpse the universe in the first trillionth of a second after the Big Bang, say proponents of supersymmetry, we would see that the forces initially acted on equal terms. Scientists are doubtful they will ever build a particle accelerator powerful enough to recreate the high-energy conditions of the universe’s birth. But experiments at existing colliders have recreated phenomena from within the first billionth of a second after the Big Bang, and they have shown that the forces do act more similarly, although not identically, at these energies. Many theorists take this as a sign that the forces were once part of a single entity called the “superforce.”
“The arrows seem to point back to a unity that may have existed among all the disparate parts of the universe,” says Greene. “It seems that in the first moments after the Big Bang there was an elegant simplicity, a grand synthesis that shattered and eventually crystallized out into the messy world that we see around us.”
Where do all the photinos, gluinos, squarks, gravitinos, charginos, zinos, and other sparticles figure in this story? They play bit parts, to say the least. Theorists suspect that few sparticles survived more than a nanosecond after the Big Bang, and they have no idea what most of them did then. In fact, their notion of these sparticles derives mainly from their efforts to mathematically accommodate the idea that nature’s forces once were unified. And what the mathematics requires is that every known particle has a supersymmetrical partner that resembles it except for being heavier and having a different spin. (A particle’s spin is an intrinsic quality related to the way it moves.)
If this strikes you as a bit too convenient, you’re not alone. Some physicists see it this way, too. Among the idea’s chief detractors is the Nobel-winning theoretician Sheldon Glashow, who once joked that supersymmetry must be right, since “half the particles have already been discovered.”
Peter Woit, a theoretical physicist who teaches at Columbia, likens supersymmetry’s adherents to Scrabble players who, disliking the letters they have been allotted, slip their hands into the bag for a few more. “The theory doesn’t identify previously unrecognized symmetries among real entities that we have in front of us,” he says. “All of its symmetries are between things we see in nature and imaginary entities. You need to regard a theory like that with some skepticism.”
Master Keys
Far-fetched as supersymmetry might seem, large numbers of theoretical physicists and mathematicians have devoted their careers to developing the theory in recent decades. And it has proved useful in addressing many other mysteries. Take the problem of the universe’s total amount of matter: scientists say there is a discrepancy between the amount of known matter and the gravitational strength of celestial bodies. That is, if our galaxy were composed only of the elementary particles we are familiar with, it would not generate enough gravity to keep our sun and three hundred billion other stars orbiting its center. The discrepancy is glaring: scientists estimate that more than 80 percent of the universe’s mass has yet to be accounted for. Dark matter, so named because it is assumed to be unobservable, has been hypothesized to account for this discrepancy, and the invisible gravitino that Parsons is chasing is considered a leading candidate to be dark matter.
“It’s enormously striking that sparticles come with the properties necessary to make them candidates for the dark matter,” says Greene. “It didn’t have to be that way. Nature doesn’t always provide us such ready-made solutions. This is a wonderful case of a hand fitting into a glove.”
Supersymmetry is also a cornerstone of string theory, which hypothesizes that particles consist of tiny loops or strings of energy that vibrate at distinct frequencies. Because string theory offers a simple explanation for the characteristics of elementary particles and their interactions with the fundamental forces, it is regarded as a leading candidate for what scientists call a theory of everything. And it makes mathematical sense only when the total number of particles gets doubled.
Call that convenient. Or consider it a sign that Parsons and his colleagues at the LHC are on the cusp of glimpsing a hidden pattern in nature with tremendous explanatory power.
“It’s like what Einstein said of his concept of general relativity,” says Greene. “It seems too beautiful to be wrong.”
It Takes a Village
More than twenty Columbia physicists are now working at the LHC, which is overseen by the European Organization for Nuclear Research, or CERN, and lies three hundred feet below ground at the French-Swiss border. The physicists include doctoral candidates like Diedi Hu and Andrew Altheimer, who specialize in analyzing gigantic data sets; postdoctoral researchers like Emily Thompson, who studies dense plumes of energy, or jets, that sometimes shoot out of particle collisions; and undergraduates like Nilay Kumar, who is a whiz at writing computer code used in physics experiments. All of the members of Columbia’s LHC team, which is led by Parsons and fellow physics professors Gustaaf Brooijmans, Emlyn Hughes, and Mike Tuts, made important contributions to the discovery of the Higgs boson. Many are now involved in the search for supersymmetry.
“This is clearly the next hot thing,” says Hughes. “Most of my graduate students now want to look for sparticles.”
Columbia physicists developed many of the electronic components inside the Large Hadron Collider’s ATLAS detector, whose eighty-foot-tall cylindrical banks of magnets and sensors are often pictured in media reports. The scientists are now responsible for maintaining the equipment they made and for helping other physicists interpret the data generated by their instruments. Many of these components were designed and tested at Columbia’s Nevis Laboratories in Irvington, New York.
“Say it’s 3:00 a.m. and there’s a question about how reliably your electronics are working,” says Nikiforos Nikiforou, a Columbia PhD student who lives and works full-time at the LHC. “You might have to get your team down into the pit and run some diagnostic tests. Your job is to do whatever is necessary to make sure that detector is working smoothly at all times.”
Today, these Columbia physicists are helping to lead a major LHC renovation and upgrade. The $10 billion machine, which has already produced collisions four times as powerful as any in the past, is now being readied for even higher-energy collisions that will take place in 2015. The scientists expect that by smashing protons at faster speeds and in greater quantities they will more than double the amount of energy the collisions release per second. This could help find evidence of supersymmetry, scientists say, because most sparticles are hypothesized to appear only at higher energy levels than the LHC has reached so far.
“The higher the energy level, the further back you’re inching toward the conditions of the Big Bang,” says Parsons. “And every bit of progress can make a difference in the phenomena you see.”
Supersymmetry is not the only concern of the Columbia physicists at the LHC. Parsons, Brooijmans, Hughes, and Tuts have graduate students who continue to analyze the Higgs boson in order to better understand how it behaves. This work will continue for years. And some of the scientists are hoping to see familiar particles do unfamiliar things. Brooijmans is studying the top quark, which is among the more exotic of the seventeen known particles, to see how it behaves when shot out of collisions at nearly the speed of light.
“The top quark is the heaviest of the seventeen known particles, which means it is the one the Higgs binds to most strongly,” says Brooijmans. “It only existed naturally in the very first moments of the universe. But at the LHC, we’re able to produce several top quarks each second. If there exist particles that are heavier than those we’ve seen, it’s probable that they would decay into the top quark or interact with it. This could provide a window into all sorts of new physics phenomena.”
All of the experimental physicists interviewed for this article expressed at least mild skepticism that evidence of supersymmetry will be found at the LHC. But the prospect of learning firsthand whether nature follows the script written in the theorists’ notepads clearly excites them.
“Honestly, it’s what’s keeping me on the project,” says Hughes. “I’m the type of scientist who has always loved solving puzzles and supersymmetry is the most amazing half-finished puzzle. If you take it off the table, the field of particle physics just wouldn’t have the same allure for me.”
Tuts, who oversees four hundred ATLAS scientists as the project’s US operations program manager, will step down from that position this fall to return to his own research. He might look for evidence of extra dimensions, a component of most versions of supersymmetry and string theory. To illustrate the mind-bending concept, Tuts offers an analogy of a person who lives on a tightrope and whose only options are to walk forward or backward. Such would be a one-dimensional existence. But what if he could shrink down to the size of an ant? Then he would realize that his rope is filled with crevices into which he can crawl. Many theorists today suggest that our universe contains up to seven extra dimensions that likewise are too tiny for us to notice.
“If this were true, you might see particles disappear without a trace, suggesting they’ve slipped into another dimension,” Tuts says. “Do I think that’s likely? Not really. I personally find the concept a bit far-fetched. But I think you have to look for it. The potential payoff is just too big.”
Lampposts and Dark Corners
Parsons has not found evidence of supersymmetry yet. The gravitino he thought he saw? He concluded it was a fluke.
This is a familiar story. In the past year, scientists at the LHC have published dozens of papers detailing their searches for sparticles. None have turned up gravitinos, selectrons, or squarks — only statistical blips, hiccups, and quirks.
Critics say this is telling. The most popular versions of supersymmetry, which are the simplest and those with the broadest implications, predicted that sparticles would be discovered almost immediately after the LHC was turned on, much sooner than the Higgs.
“Am I surprised that no evidence of supersymmetry has been found?” says Woit. “No. And if it isn’t found soon, the best outcome would be for theorists and experimentalists to shift their focus elsewhere. There are plenty of other questions they could be working on. We’ve only just begun to understand how the Higgs works. That could keep them busy for years.”
Few physicists have ruled out supersymmetry altogether. Some, including Parsons, say there remains a small possibility that sparticles could still be found in data that was produced before March, when the LHC was shut down temporarily to prepare for higher-energy experiments. A more likely scenario, he and other physicists say, is that sparticles will be found in 2015, when the LHC finally fires on all cylinders.
“There is a growing realization that if supersymmetry exists, it probably isn’t the version we expected,” says Parsons. “The question now is whether or not the entire concept is invalid.”
How long could the search for supersymmetry go on? Could it last forty years, like the search for the Higgs? This is, in fact, the great fear of many physicists. They worry that the LHC won’t find evidence of supersymmetry and yet won’t disprove it either, since it can always be argued the collider simply isn’t powerful enough to see sparticles. This could put the field in limbo, with some theorists spinning off what University of Minnesota physicist Mikhail Shifman recently warned would be “contrived, baroque-like, aesthetically unappealing modifications” of supersymmetry instead of breathing new ideas into the field.
“I would be thrilled if we could rule out supersymmetry and string theory,” says Greene. “People might think that sounds odd, since I’ve spent my professional life working on these ideas, but I’m not wedded to any theory. What I want is to find out something true about nature. Unfortunately, if no evidence comes through at the LHC, it won’t necessarily mean these theories are wrong.”
In the meantime, Parsons continues to run his analyses, looking for unusual photonic activity. To do this work, he logs on to the LHC’s website, downloads a few gigabytes of data to his laptop from a gymnasium-sized computer center in Switzerland, and runs statistical-analysis programs that he and his students created. He does this work on the subway, at home in his pajamas, and at the ice rink in suburban New Jersey where he plays hockey every Thursday night. Most days he does it in his office, a drab and sparsely decorated place littered with cardboard boxes — evidence of his constant travels between Morningside, Nevis Labs, and Geneva.
“If the gravitino exists, it’s good at hiding,” he says. “The only way to find it is to be patient. We’ve looked under the lampposts. Now we need to look in the dark corners.”