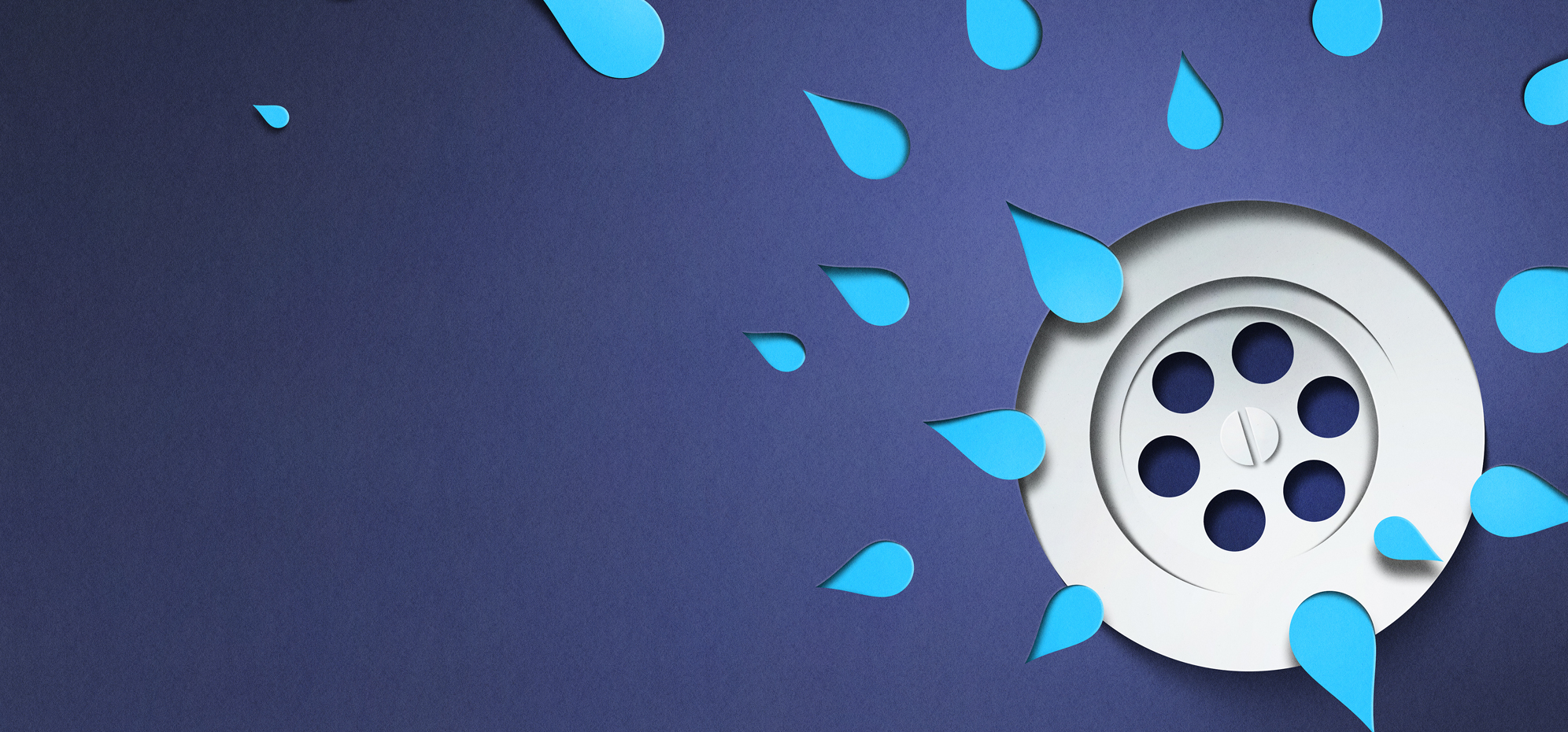
Gone Fishing
Luke Plante, a twenty-eight-year-old Army captain dressed in jeans, a white T-shirt, and size 11½ steel-tipped work boots, opens the back of his white Ford Escape. He takes out a red Rubbermaid cooler and sets it down on the gravel under the glaring morning sun. “This’ll be quick,” he says.
He snaps on a pair of latex gloves, then picks up the cooler and carries it to the water’s edge.
The water is grayish-brown, fifteen feet deep. It sits in a narrow tank more than a hundred yards long. There are twelve of these tanks in a row. Divided by strips of cement walkway, they resemble individual swimming lanes. The air smells of ammonia, with an undercurrent of some grim, dank putrefaction.
Yet the water rushing into the complex from the city’s sewer pipes makes a soothing whisper, and there is a coolness to be drawn along the cement pathways, like the coolness near a pond on a hot day.
Plante has come to this wastewater-treatment facility in eastern Brooklyn (one of fourteen in New York City) as a graduate student in environmental engineering at Columbia. It’s his fourth visit this year.
In some tanks, the water churns. These tanks are being pumped full of air — a costly and energy-intensive process — to sustain the greedy bacteria that reside there. The bacteria’s dietary habits lower the nitrogen levels in the water before it gets released into the nearby creek. Nitrogen promotes the growth of algae, which suck up oxygen and block light, resulting in fish kills and dead zones.
But the water at which Plante stops is calm. No aeration in this tank. No oxygen, period. This water has what he needs.
Plante sets the cooler down on the cement and picks up a wooden pole with a plastic cup taped to one end. The water at his feet is murky, but if you look closely, you can see, floating here and there — and there, and there — cotton-ball-sized clumps of soggy brownish matter, tinged with red.
Guiding the pole, Plante dips the plastic cup into the water, adroitly scoops up some of the red stuff, and dumps it in the cooler. He does this five times. Then he closes the cooler, seals it with duct tape, wipes it down, and carries it back to the car.
It’s an hour’s drive to Morningside Heights. Plante parks the car, grabs the cooler, and walks across campus to the Mudd Building and the laboratory of Kartik Chandran, an associate professor of earth and environmental engineering at Columbia.
Now the real work begins.
The Gray and the Black
How much water do you think is reused in the United States?” says Kartik Chandran, seated in his office next door to his laboratory in Mudd.
Chandran has short, dark hair and wears rectangular glasses. At forty, he is one of the world’s top experts on recovering clean water and resources from sewage, and before he answers his own question he sets down some basics: wastewater is classified either as graywater (from sinks, tubs, washing machines) or blackwater (from toilets); domestic wastewater contains levels of nitrogen, carbon, and phosphorus — mainly from urine, feces, food, soap, and detergent — that can harm aquatic ecosystems; Americans use, on average, a hundred gallons of fresh water a day per person; a quarter of the US water supply comes from underground aquifers; a majority of aquifers are being drained faster than they are being refilled.
“The US reuses 7 percent of its water,” Chandran finally says. “Next question: how much water do you drink a day?”
Hmm. Let’s see: there’s wake-up coffee, mid-morning coffee, a glass of water with lunch — “Half a gallon?” says Chandran. “Let’s say half a gallon a day.”
Sounds reasonable.
“So,” says Chandran, “if we each drink half a gallon per day out of a hundred gallons used, we are drinking only one two-hundredth of what we consume. Yet we are treating all our incoming water to drinking-water quality. This makes no sense. Using potable water for everything — for irrigating crops, for flushing the toilet, for washing your car — takes more energy, more chemicals, more space. It’s needless.”
Our outflow is similarly mismanaged, says Chandran: he rejects the notion of “waste” with regard to human effluvia. “It’s not wastewater, it’s used water,” he likes to say. And it’s valuable, too: “Used water has higher energy — it’s enriched compared to just H2O.”
There is gold in those streams, so to speak.
“In a healthy person, urine is near-sterile — it is mostly nutrients,” Chandran says. “Why do we need to treat it to near-drinkable levels and then use it for irrigation? Why not take it right to the fields? Since urine already contains nitrogen and phosphorus — the nitrogen is in the form of ammonia — this water could be directly used for irrigation. The nutrients are there in the form that plants need them. How do we currently get fertilizer? We spend horrendous amounts of energy converting dinitrogen gas into ammonia. Yet we have ammonia right here, and instead of using it, we use more energy to get rid of it.”
Seen through Chandran’s goggles, municipal effluent is a sea of energy and nutrients waiting to be ingeniously tapped, an efficient way to lower energy costs, save resources, and reduce greenhouse emissions. The clean water that comes out at the end? That, Chandran says, is “the bonus.”
“This is not a conversation about water alone anymore,” he says. “This is about water sustainability. And one way to water sustainability is to stop talking about just water.”
Pumping Up the Volume
Two floors below Chandran’s lab, at the Columbia Water Center — a research consortium of scientists and engineers — they spend their days talking about water. Yet if you listen closely, you realize that they aren’t talking about water alone. They’re talking about water’s children: food, energy, climate, cities. They, too, speak the language of sustainability.
“Fresh water is a finite resource,” says Upmanu Lall, the Alan and Carol Silberstein Professor of Engineering and the Water Center’s director. As Lall explains, Earth’s hydrologic cycle is a constant exchange between the seas and the atmosphere: ocean water evaporates; the H2O molecules disperse in the air, drift, condense, and fall as precipitation; this water, should it fall over land, evaporates or is absorbed by plants (which return it through transpiration, a vapor from leaves) or locked in soil or caught in lakes, rivers, and reservoirs (surface water) or filtered through the soil to aquifers (groundwater). The rest returns to the ocean. Water isn’t gained or lost: it only changes form and location. The water in your teacup is the water Brontosaurus bathed in. We have what we have.
Now consider that 97 percent of Earth’s water is salt water. The rest is fresh water, of which more than two-thirds is frozen in ice sheets. The remainder — 1 percent of all the water on the planet — is surface water (those lakes and rivers), or groundwater. This is our water supply. Last year, the Water Center released a report showing that most aquifers in the US are being depleted, and a study this summer from the University of California at Irvine used data from satellites that indicate stress in twenty-one of the planet’s largest thirty-seven aquifers. When groundwater runs out, it can take years, even centuries, for it to be restored.
In the US, the trouble has been most conspicuous in California’s Central Valley, a major agricultural region.
“We’re pumping groundwater that, due to drought, isn’t being replenished,” says Michelle Ho, an engineer and Water Center postdoctoral research scientist. “In some cases, heavily pumped aquifers have collapsed — the ground caves in — and there’s no chance of water ever being stored there again.”
Ho, who grew up in bone-dry Australia, notes that around 70 percent of water that humans use goes to agriculture.
“Everything on your plate needs water,” she says. “Most people don’t realize how much water goes into making that nice steak.” (Answer: about 1,200 gallons for eight ounces of beef.) “Look in your fridge, look in your closet.” (Nine hundred gallons for a pair of jeans.) “We need to focus on crop management and find market-based solutions to help the agricultural sector use water wisely.”
Michael Puma ’99SIPA, a Water Center affiliate who studies the hydrologic cycle in relation to climate, ecosystems, and society, agrees. “In a democratic country with a market economy — farmers grow crops in response to the market — it’s much more difficult to start mandating what to grow or not grow,” says Puma, who teaches in the sustainability-management program at the School of Continuing Education. “You don’t want people who have never been out in the field to bring in the farmers and say, ‘Oh, great, you’re here. Now we’re going to tell you what to do.’ That’s not going to work. We need state-level efforts to improve management of scarce water resources, and to find ways to optimize water use through the selection of crops grown in a given state. But this has to be done together with the farmers.”
Puma also shares Ho’s concern about groundwater: from California to the Ogallala Aquifer beneath the US Great Plains, this indispensable element is being tapped to its ancient depths. The deeper you drill, the older the water.
“This water was deposited there thousands and thousands of years ago,” Puma says. “Farmers rely on that groundwater. But it’s not a resource that’s going to last.”
The Magical Microorganism
Inside Chandran’s lab, the lid comes off the Rubbermaid cooler.
The lab is filled with pitcher-sized bioreactors containing bacteria whose chemical-altering capabilities Chandran wants to understand. He and his students are working on ways not only to capture and reuse the nitrogen, carbon, and phosphorus in wastewater, but also to remove modern impurities like antibiotics and hormones (from factory farms) and pharmaceuticals (from your medicine cabinet). The bioreactors, built by students, hum and beep continuously, and have a homespun science-fair quality, basic yet sophisticated, their tubes suggestive of a high-tech bong. In one of them floats a substance of copper-red.
Though it has been around for billions of years, the red stuff is a newcomer to the reservoir of human knowledge, and is the answer to one of the great mysteries in the history of sewage treatment. Typically in the nitrogen-removal process, bacteria in aerated tanks consume nitrogen (in the form of ammonium), convert it to nitrite, then to nitrate, and lastly to nitrogen gas, which then bubbles from the tanks and diffuses harmlessly in the air. (Nitrogen makes up 79 percent of the earth’s atmosphere and more than 60 percent of all ocean gases.) But in some baffling instances, ammonium has appeared to be converted directly to nitrogen gas, without any of those energy-sucking intervening steps — a sort of alchemy with no known biological basis. It wasn’t until the early 1990s that researchers at Delft University of Technology in the Netherlands finally identified the process by which certain aquatic bacteria turn ammonium straight to nitrogen gas — without the need for pumped-in air.
Scientists named the process “anaerobic ammonia oxidation,” which was abbreviated and trademarked as the Seussian-sounding “anammox.” Anammox bacteria, like the ones from Plante’s cooler, are recognizable by their redness, which comes from their peculiar enzymes.
Chandran is a leader in anammox research. Before he came to Columbia in 2005, he worked in an engineering firm responsible for redesigning New York’s water-treatment systems to go from just carbon removal to nitrogen removal as well. Now his lab is part of an Environmental Protection Agency center focusing on sustainable nutrient management, and Chandran receives anammox bacteria and other bacteria from water utilities all over the world. He and his group take the samples and interrogate their biology: Are the bacteria doing what they’re supposed to be doing? What sort of bacteria are there, and how many? What are the concentrations? Are they the best bacteria for the job? How can they be made better?
“We are developing anammox processes that consume anywhere from 25 to 60 percent less energy and eliminate nearly all CO2 emissions,” says Chandran. “We are removing nitrogen at a far lower carbon footprint, while also generating nutrients and energy.”
Chandran doesn’t limit himself to the liquid side of waste. He has also pioneered a biochemical method to convert the methane from fecal sludge into methanol (methanol is used in wastewater-treatment plants to convert nitrite and nitrate into nitrogen gas). “The way we do things now is, we take organic matter, produce methane from it, and flare the methane we don’t use,” Chandran says. “Then we spend a hundred million dollars per year purchasing methanol. So my group has connected methanol into the process. No flaring, no buying methanol. We can produce the methanol internally.” For this, Chandran won the 2010 Paul L. Busch Award, a $100,000 research grant from the Water Environment Research Foundation.
“Kartik has a unique approach to bioengineering,” says Liron Friedman, a PhD candidate at the Water Research Center of Tel Aviv University who works in Chandran’s lab as part of his doctoral program. “He doesn’t look at bacteria according to species. He looks at function. He wants to see what they do. What are the functional abilities of the community? That’s what I’m doing: looking at bacteria as groups that can do something for the water. I’m manipulating the bacteria, using more nutrients, less nutrients, and different oxygen sources, seeing how that affects the community. I’m looking for groups that can take compound A and turn it into compound B. How many are there? How efficient are they? How fast? I’m an engineer: I want it better, faster, more efficient.”
At Columbia, Friedman is experimenting with a system he worked on in Israel, an arid country that recycles 80 percent of its water. The main Israeli process uses sand as a bioactive filter: water passes through the bacteria-rich sand for about a year before going straight into an aquifer. The chief machinery is gravity. “It takes less energy, and no special membranes or tubes or chemicals,” says Friedman. “Just pump the water out, and that’s it. This water is very high-quality, and it’s used for irrigation.”
Friedman is simulating this field process in Chandran’s lab, working on a controlled sand-based system that turns wastewater-treatment-plant effluent into water that can be safely reused.
“Look, this is the future,” Friedman says. “Water scarcity is here, and it’s already the first resource that’s in severe shortage in the world. Right now, there’s enough oil, enough coal, enough aluminum, enough natural gas — but there’s not enough fresh water in the world to supply everyone.”
Flush with Possibilities
Upmanu Lall once worked as a hydrologist in Utah, and he has intimate knowledge of the water ways of the West.
Take flood irrigation, still common in California. “Flood irrigation is low-efficiency,” Lall says. “Think of the backyard garden: typically, people use a sprinkler. The water goes into the ground, into the root zone, and is used by the plant. In flood irrigation you have a lot of water just sitting on the surface. Fifteen to 20 percent will evaporate under action of wind and radiation, and it’s lost. It’s gone. It had no productive capacity. Sprinklers can significantly increase efficiency. Drip irrigation is even better, but only for specific crops like grapes and walnuts.”
Lall is also critical of what he sees as excessive energy use. He points to the biggest expense in the California system: the power needed to pump water from central California over the mountains and into Southern California.
“The energy use connected with that pipeline is even greater than that of building a desalination plant,” Lall says. (Desalination plants, which cost hundreds of millions of dollars to build, burn vast amounts of fossil fuels.) “In the California legislature, some people are pushing for a second pipeline. This requires three times the energy needed to treat wastewater to drinking-water standards. The smarter thing would be to take wastewater and super-treat it so you are back to H2O level.”
This is the nose-wrinkling idea that’s been branded in the media as “toilet to tap.”
Lall acknowledges that “for most people there is still an ick factor,” but necessity has a way of eroding psychological barriers: localities in California and Texas are already recycling blackwater for drinking, through further purification steps like UV-light exposure and the energy-hungry filtration method of reverse osmosis.
Closer to home, Lall, like Chandran, sees waste of a different color: green.
“I live in Manhattan, I have no lawn, and my water bill is $120 a month,” he says. “One-fourth for delivery, and three-quarters for cleaning the wastewater I generate. What do we do with the wastewater? We treat it and dump it into the Hudson. I’m paying three times the cost of my drinking water to dump it into the Hudson. Toilets, washing machines, showers: why are we treating it all to the same standard?”
Pipe Dreams
Last May, in East Bridgewater, Massachusetts, a two-foot-wide water main broke. The rupture of the decades-old pipe, as reported by the Boston Globe, affected seventy-five thousand people, “shuttering businesses, forcing the cancellation of surgeries at a hospital, and closing schools,” and leaving whole towns with little or no water.
This is not unusual. At any given moment in the US, pipes break, streets and highways are inundated, basements flood, roadways buckle, water-boiling advisories are issued, service is cut off, and life changes at its most basic level.
“In many locations, our water-delivery systems are reaching the end of their design lives,” says Michelle Ho. “In the US, 20 percent of water is lost through pipe leakage. We can look at that as a waste, but we can also look at it as an opportunity to totally overhaul how we deliver and manage water.”
Kartik Chandran dreams of such a makeover.
In the dream, Chandran sees pipes. Not one pipe, like the kind that brings treated, potable water to our homes and businesses, so that we wash our cars and soak our lawns with drinking water; nor like the lone sewer pipe that mixes graywater and blackwater together on its way to the wastewater-treatment facility, where the fluid must be treated to a single rigorous standard.
No: Chandran sees dual pipes.
“Two pipes in, two pipes out,” he says.
In: water for toilets, and water for everything else (drinking, cooking, washing).
Out: graywater and blackwater, to separate destinations.
“Graywater can be treated and used for non-potable purposes, like for cooling power plants,” Chandran says. “If you separate the water, you’re super-treating not one hundred gallons per person per day, but maybe ten gallons. So these massive plants can be one-tenth the size. Then, instead of using more energy to convert the carbon in the water into CO2 and then releasing it into the atmosphere — a stupid thing to do — you can produce energy from it and run the plant on that energy.
“Now,” says Chandran, “you can put these plants in buildings. In Mudd we have a plant where we’re recovering carbon in food waste for biodiesel. Now we can talk about nutrient recovery, and urban farming on campus: we can take food waste from our cafeteria, get energy out, get clean water out, get the nutrients, and grow crops for food.
“Come, let’s go outside — I will show you something.”
It’s a sunny summer day. Out on Pupin Plaza, behind the curved rear of Uris Hall, two green plots of land the size of motel kiddie pools are abuzz with herbage and nectar-fueled bees. There are shocks of green grass, yellow-buttoned daisies, blue cones of delphinium, stalks of metallic-green Swiss chard veined bright crimson, heirloom tomato plants chock-full of dangling pale-green fruit. Against a brick wall lean a shovel, a hoe, and a faded painted sign that says “Food Sustainability Project.”
This is the Columbia Community Garden. During the spring of 2011, Chandran’s engineering students, who were using the food waste from the Mudd cafeteria to produce biodiesel and methane, worked to recover nutrients as well, which they used as fertilizer to grow food in this garden. It was a glimpse of a system of resource recovery and reuse that Chandran envisions on different scales: home, building, city. He mentions residential buildings like the Visionaire in Lower Manhattan, which repurposes graywater for the cooling tower, toilets, and green-roof irrigation.
“This isn’t science fiction,” says Chandran, and you get the feeling he’s talking about not just these solutions, but also the crises occasioning them. “This is already happening.”
Ripple Effect
Once he gets his master’s degree, Luke Plante will return to his alma mater, West Point, and teach in the engineering department from which he graduated. Plante knows the value of water infrastructure, having spent time in Afghanistan, where the rates of access to safe drinking water are among the lowest in the world.
On the morning of his anammox rendezvous in Brooklyn, Plante, the cooler in his grip, pauses to observe the slender channel of water that runs adjacent to the facility.
This seven-thousand-foot-long tributary, straight as a landing strip, flows into Jamaica Bay. The creek used to be fed by a stream. Now it receives the nitrogen-reduced, chlorine-treated outflow of the wastewater-treatment plant, one of the five New York facilities that Chandran had worked on.
On the other side of the water is a big-box-store shopping center (Best Buy, Marshalls, BJ’s Wholesale Club), but Plante’s eyes are trained on the silvery creek itself, which, bordered by the wastewater plant and the shopping complex, wears an aspect of challenged ecology, of uncertain habitat.
An outcropping rises spine-like in the middle of the creek, and on it stands a big, long-necked, shimmery black bird. A great cormorant? The bird is perfectly still.
There’s a splash in the water.
“A fish!” says Plante, pointing at the wrinkles on the surface, where a fleck of orange appears and quickly vanishes. “That was a fish.”
Plante shields his eyes and watches the water a little while longer. The sun climbs a stairway of dense white clouds. Water cascades into the creek, incessant as a waterfall, and rolls slowly to the bay.
Kartik Chandran is an associate professor of earth and environmental engineering at Columbia. Applications of his work include several full-scale wastewater-treatment plants around the globe and biorefineries in North America and sub-Saharan Africa.